Introduction:
DNA damage is a natural occurrence that happens constantly in living cells. It can be caused by a variety of factors, including environmental agents such as radiation and toxins, as well as the natural processes of metabolism and aging. DNA damage can take many different forms, such as base modifications, strand breaks, crosslinks, and DNA adducts, and it can have serious consequences for the function of cells, tissues, and organs.
To protect against the harmful effects of DNA damage, cells have evolved a variety of DNA repair mechanisms. These mechanisms work to identify and repair damaged DNA, ensuring that the genetic information carried by DNA is maintained accurately.
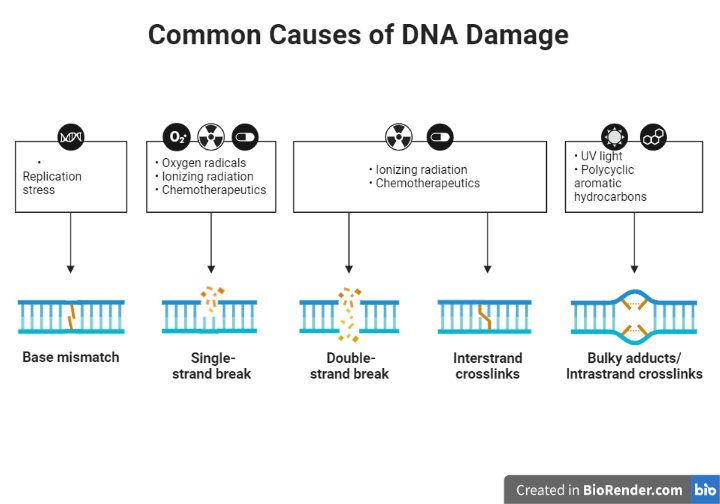
Fig: Common Causes of DNA Damage
DNA repair is a process by which cells identify and fix damage to the DNA molecule. DNA damage can occur as a result of various factors, including exposure to environmental agents such as UV radiation and certain chemicals, errors during DNA replication, and normal metabolic processes. If left unrepaired, DNA damage can lead to genetic mutations and increase the risk of cancer and other diseases.
DNA repair mechanisms in cells help to maintain the integrity of the genetic material and prevent the accumulation of genetic mutations that could lead to diseases such as cancer.
Types:
Direct repair mechanisms
These mechanisms repair DNA damage directly by reversing the damage or by synthesizing a new stretch of DNA to replace the damaged region. Examples of direct repair mechanisms include:
Base excision repair
This mechanism repairs small base lesions or base modifications in DNA by removing the damaged base and replacing it with a new one. The process begins with the recognition of the damaged base by specialized proteins called DNA glycosylases. These proteins remove the damaged base by hydrolysing the bond between the base and the sugar phosphate backbone of DNA. The resulting abasic site is then repaired by a group of enzymes called AP (apurinic/apyrimidinic) endonucleases, which cleave the sugar phosphate backbone at the abasic site. The resulting gap is filled in by DNA polymerase and ligase, which synthesize a new stretch of DNA and join it to the rest of the molecule.
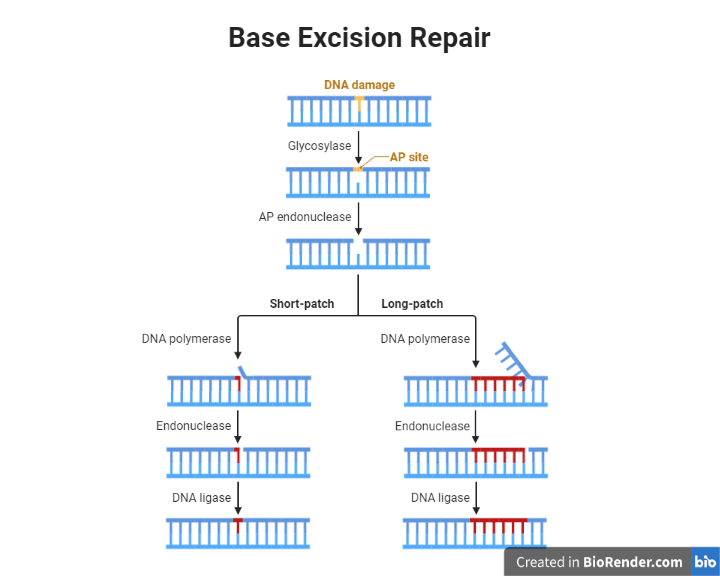
Fig: Base Excision Repair
Recognition of the damaged base: DNA glycosylases, such as OGG1 and MYH, recognize the damaged base and remove it by hydrolysing the bond between the base and the sugar phosphate backbone.
Cleavage of the sugar phosphate backbone: AP endonucleases, such as APE1 and APN, cleave the sugar phosphate backbone at the abasic site, creating a gap in the DNA molecule.
Filling in the gap: DNA polymerase synthesizes a new stretch of DNA using the undamaged template, and ligase joins it to the rest of the molecule.
Nucleotide excision repair
This mechanism removes a stretch of DNA that contains a damaged base or a bulky adduct. The process begins with the recognition of the damaged DNA by specialized proteins called transcription-coupled repair factors. These proteins bring in other repair proteins, including helicases, which unwind the double helix to expose the damaged DNA. The damaged region is then removed by a group of enzymes called nucleases, which cut the sugar phosphate backbone on either side of the damaged region. The resulting gap is filled in by DNA polymerase and ligase, which synthesize a new stretch of DNA and join it to the rest of the molecule.
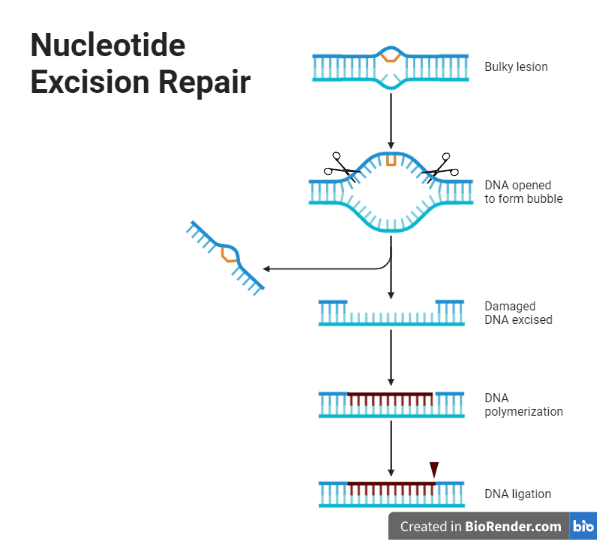
Fig: Nucleotide Excision Repair
Recognition of the damaged DNA: Transcription-coupling repair factors, such as XPC, bind to the damaged DNA and recruit other repair proteins to the site.
Unwinding of the double helix: Helicases, such as RecG and UvrD, unwind the double helix to expose the damaged DNA.
Removing the damaged DNA: Nucleases, such as ExoI and EndoIII, cut the sugar phosphate backbone on either side of the damaged DNA, removing it and a short stretch of DNA on either side of it.
Filling in the gap: DNA polymerase and ligase synthesize a new stretch of DNA using the undamaged template and join it to the rest of the molecule
Mismatch repair
This mechanism corrects errors that occur during DNA replication, such as mismatched base pairs. The process begins with the recognition of the mismatch by specialized proteins called mismatch recognition proteins. These proteins bring in other repair proteins, including helicases and nuclease, which remove the mismatched base pair and a short stretch of DNA on either side of it. The resulting gap is filled in by DNA polymerase and ligase, which synthesize a new stretch of DNA and join it to the rest of the molecule.
bind to the mismatched base pair and recruit other repair proteins to the site.
Unwinding of the double helix: Helicases, such as RecG and UvrD, unwind the double helix to expose the mismatched base pair.
Removing the mismatched base pair: Nucleases, such as ExoI and EndoIII, cut the sugar phosphate backbone on either side of the mismatched base pair, removing it and a short stretch of DNA on either side of it.
Filling in the gap: DNA polymerase and ligase synthesize a new stretch of DNA using the undamaged template and join it to the rest of the molecule.
Recombinational repair mechanisms
Recombinational repair mechanisms are a group of DNA repair mechanisms that repair DNA damage by using undamaged DNA sequences as a template for repair. This process involves the exchange of genetic material between two DNA molecules, and can occur either between sister chromatids (homologous recombination) or between non-sister chromatids (non-homologous end joining).
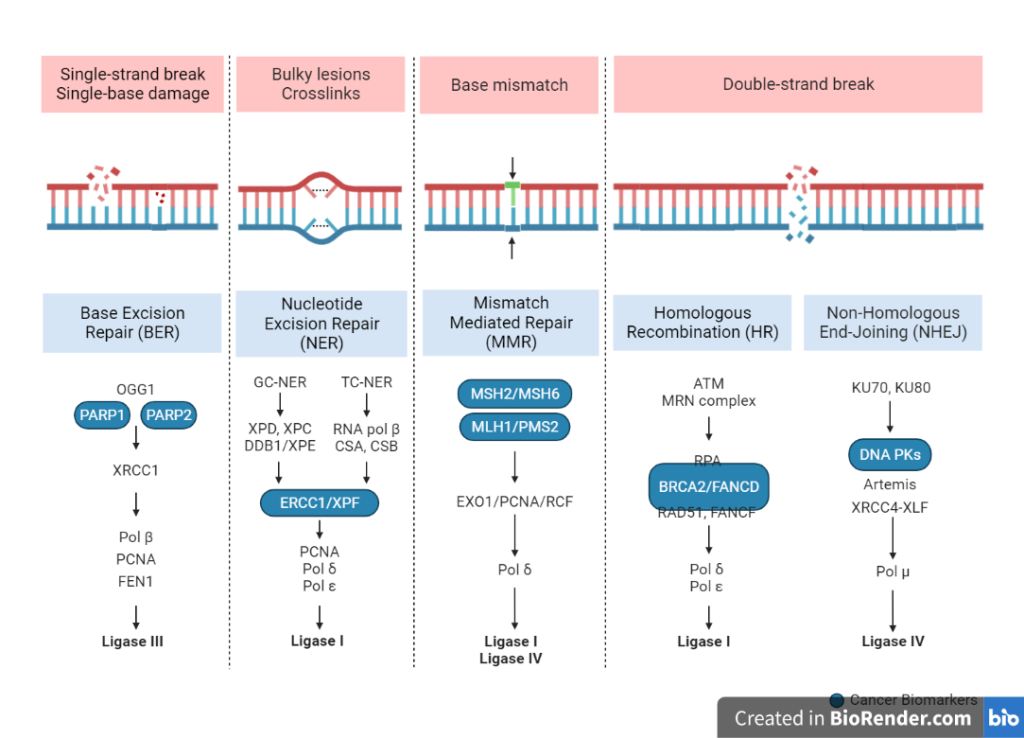
Fig: Overview of DNA repair mechanisms
Homologous recombination
This mechanism repairs DNA damage by using undamaged DNA sequences as a template for repair. The process begins with the recognition of the damaged DNA by specialized proteins called recombinases. These proteins bring in other repair proteins, including helicases, which unwind the double helix to expose the damaged DNA. The undamaged DNA is then used as a template to synthesize a new stretch of DNA, which is then joined to the rest of the molecule. Homologous recombination is a more precise mechanism that occurs during meiosis (the process of cell division that produces gametes).
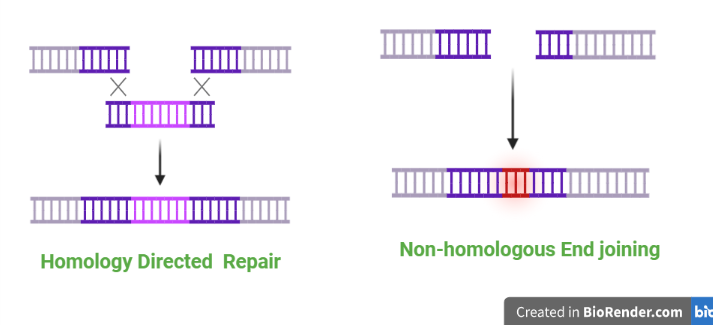
Fig: Homologous and non- homologous recombination repair mechanisms
Non-homologous end joining
This mechanism repairs DNA damage by joining the ends of two broken DNA strands together. The process begins with the recognition of the broken DNA strands by specialized proteins called Ku proteins. These proteins bring in other repair proteins, including ligase, which join the ends of the broken strands together. This process is less precise than homologous recombination and may result in the loss or insertion of a few base pairs. Non-homologous end joining is a less precise mechanism that occurs during DNA damage repair in somatic cells (non-gamete cells).
Photoreactivation Repair mechanism
Photoreactivation is a DNA repair mechanism that repairs DNA damage caused by UV radiation. UV radiation can cause a variety of DNA lesions, including cyclobutane pyrimidine dimers (CPDs) and 6-4 photoproducts. These lesions can block DNA replication and transcription, leading to genetic mutations and an increased risk of cancer.
Photoreactivation involves the enzyme photolyase, which is activated by visible light (wavelengths between 400 and 500 nm). Photolyase recognizes the DNA lesion and uses the energy from visible light to repair it by breaking the covalent bond between the damaged bases. The process is reversible and does not require a template, making it a direct repair mechanism.
Photoreactivation is most effective in repairing CPDs, but is less effective at repairing 6-4 photoproducts. Other DNA repair mechanisms, such as nucleotide excision repair, are used to repair 6-4 photoproducts and other types of DNA damage caused by UV radiation.
Overall, photoreactivation is an important DNA repair mechanism that helps to protect cells from the damaging effects of UV radiation. Defects in photoreactivation can increase the risk of cancer and other diseases.
Regulations:
DNA damage sensors: DNA damage sensors are proteins that recognize and bind to specific types of DNA damage, such as double-strand breaks or base lesions. These sensors trigger the activation of downstream signaling pathways that initiate the repair process.
DNA repair enzymes: DNA repair enzymes are proteins that catalyze the repair of specific types of DNA damage. These enzymes are typically regulated at the level of transcription, with their expression being upregulated in response to DNA damage.
Post-translational modification: Many DNA repair enzymes are subject to post-translational modifications, such as phosphorylation or acetylation, which can regulate their activity. For example, certain kinases or phosphatases may be activated or inhibited in response to DNA damage, leading to the activation or repression of DNA repair pathways.
DNA damage checkpoints: DNA damage checkpoints are signaling pathways that are activated in response to DNA damage and help to coordinate the repair process. These pathways can halt the cell cycle, allowing time for the repair of the DNA damage, or trigger cell death if the damage is too severe to repair.
Applications:
Cancer research: DNA repair defects can increase the risk of cancer, and understanding the mechanisms of DNA repair can help to identify new targets for cancer therapies. For example, drugs that inhibit DNA repair pathways may be effective at killing cancer cells that rely on those pathways for survival.
Genetic testing: DNA repair defects can cause genetic disorders such as xeroderma pigmentosum (XP), a rare condition that causes extreme sensitivity to UV radiation. Genetic testing can identify individuals with DNA repair defects and help to diagnose and manage these conditions.
Biotechnology: DNA repair enzymes are used in a variety of biotechnological applications, including DNA sequencing, gene editing, and DNA synthesis. For example, DNA polymerases, which are involved in DNA repair, are used in the polymerase chain reaction (PCR) technique, which amplifies specific DNA sequences for use in various applications.
Environmental protection: DNA repair mechanisms can be affected by exposure to environmental agents such as UV radiation, chemicals, and radiation. Understanding the mechanisms of DNA repair can help to identify and mitigate the harmful effects of these agents on human health and the environment.
Limitations:
- DNA repair is not always accurate: DNA repair mechanisms can sometimes introduce errors into the genetic material, leading to genetic mutations. For example, non-homologous end joining, a DNA repair mechanism that joins the ends of two broken DNA strands together, can result in the loss or insertion of a few base pairs. These errors can accumulate over time and increase the risk of diseases such as cancer.
- DNA repair is not always efficient: DNA repair mechanisms can sometimes be overwhelmed by the amount of DNA damage that occurs, leading to the accumulation of unrepaired lesions. For example, exposure to high levels of UV radiation can cause more DNA damage than the cell’s DNA repair mechanisms can repair, leading to the formation of genetic mutations.
- DNA repair is not always possible: Some types of DNA damage, such as double-strand breaks, are difficult or impossible to repair. If these types of damage are not repaired, they can lead to the loss or rearrangement of genetic material, which can have serious consequences for the cell.
References:
- David, L., Nelson, D.L., Cox, M.M., Stiedemann, L., McGlynn Jr, M.E. and Fay, M.R., 2000. Lehninger principles of biochemistry.
- Ceccaldi, R. et al. 2016. Repair Pathway Choices and Consequences at the Double-Strand Break, Trends in Cell Biology, 26, pp. 52-64.
- Dexheimer, T.S., 2013. DNA repair pathways and mechanisms. In DNA repair of cancer stem cells (pp. 19-32). Springer, Dordrecht.